Drone Flight Theory: Physics and Principles of Multirotor Flight
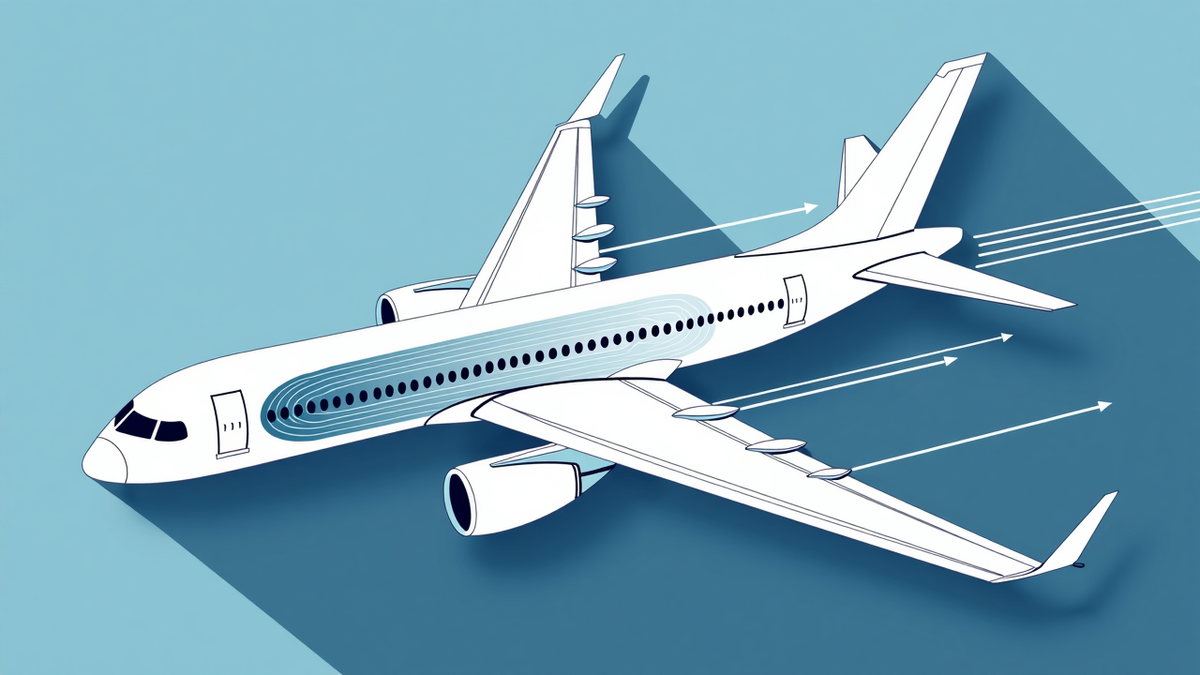
Understanding the theoretical principles behind drone flight is essential for pilots who want to master their craft, troubleshoot problems, and optimize performance. After years of studying flight dynamics and building countless drones, I've found that a solid grasp of flight theory can transform a good pilot into an exceptional one. This comprehensive guide will walk you through the physics and principles that make multirotor flight possible.
Introduction to Multirotor Flight Theory
Multirotors represent a unique category of aircraft that differ fundamentally from traditional fixed-wing planes and helicopters. Their ability to hover, maneuver precisely, and transition between various flight modes makes them versatile but also introduces complex flight dynamics.
The Fundamental Difference
Unlike fixed-wing aircraft that generate lift through airfoils moving through the air, or traditional helicopters that use complex mechanical systems like swashplates to control a main rotor:
- Multirotors use multiple fixed-pitch propellers
- They achieve control through differential thrust between motors
- They lack the mechanical complexity of helicopters but require sophisticated electronic control systems
This approach creates a mechanically simpler but electronically and mathematically more complex flight system.
Basic Physics of Multirotor Flight
The Four Forces
Like all aircraft, multirotors are subject to four primary forces:
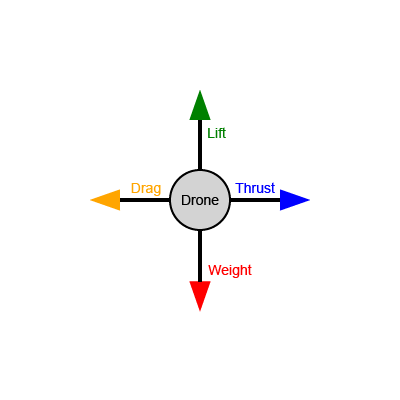
- Lift: The upward force generated by the propellers
- Directly proportional to the square of propeller RPM
- Affected by propeller design, air density, and motor power
- Can be vectored (directed) by tilting the drone
- Weight: The constant downward force due to gravity
- Equal to the mass of the drone multiplied by gravitational acceleration
- Acts through the center of gravity
- Remains constant regardless of drone orientation
- Drag: The resistance force opposing motion through air
- Increases with the square of velocity
- Affected by the drone's aerodynamic profile
- Acts in the opposite direction of motion
- Thrust: The force that propels the drone horizontally
- Generated by redirecting propeller force through tilting
- Allows forward, backward and lateral movement
- Must overcome drag to maintain horizontal motion
Additional consideration:
- Inertia: While not one of the four fundamental forces, it affects drone dynamics
- Determined by the drone's mass and its distribution
- Affects how quickly the drone can change direction or speed
- Higher mass means greater stability but reduced agility
Thrust Generation
The fundamental equation for thrust generated by a propeller is:
T = CT × ρ × n² × D⁴
Where:
- T = Thrust (N)
- CT = Thrust coefficient (depends on propeller design)
- ρ = Air density (kg/m³)
- n = Rotational speed (revolutions per second)
- D = Propeller diameter (m)
This equation reveals several important insights:
- Thrust increases with the square of rotational speed
- Thrust increases with the fourth power of propeller diameter
- Larger props are significantly more efficient than smaller ones
- Air density affects thrust (performance decreases at higher altitudes)
I've observed this relationship firsthand when testing different prop sizes. Moving from a 5" to a 6" propeller (a 20% increase in diameter) can theoretically provide a 107% increase in thrust at the same RPM, though real-world gains are typically lower due to motor limitations.
Multirotor Configuration and Control Principles
Common Configurations
Multirotors come in various configurations, each with unique characteristics:
- Tricopter: Three motors, requires a servo for yaw control
- Quadcopter: Four motors, the most common configuration
- "X" configuration (motors form an X pattern)
- "+" configuration (motors form a + pattern)
- Hexacopter: Six motors, greater lifting capacity and redundancy
- Octocopter: Eight motors, maximum lifting capacity and redundancy
The quadcopter has become the standard for most applications due to its optimal balance of simplicity, efficiency, and control authority.
Motor Rotation Patterns
For a multirotor to maintain stable flight, motors must rotate in opposing directions to counteract torque effects:
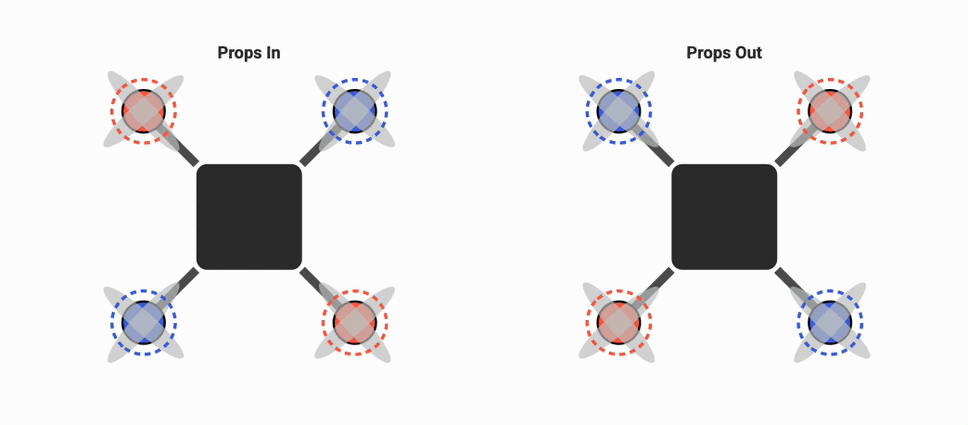
In a typical quadcopter:
- Diagonal motors rotate in the same direction
- Adjacent motors rotate in opposite directions
- This pattern balances torque forces during hover
- It also provides the necessary control authority for maneuvers
The Six Degrees of Freedom
Multirotors operate with six degrees of freedom (6DOF), which represent all possible ways the aircraft can move in three-dimensional space:
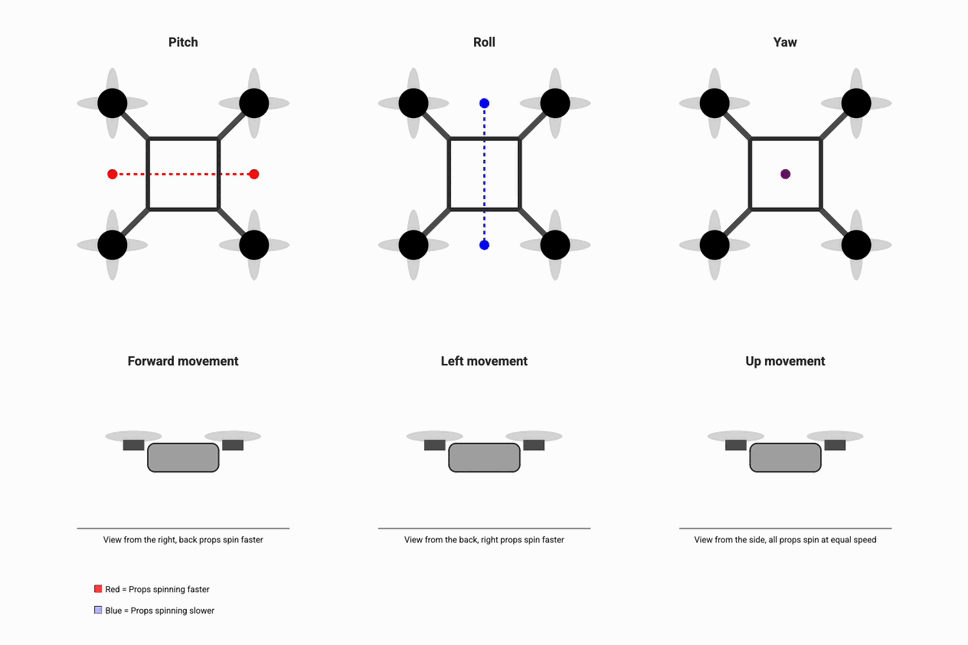
- Translation along three axes:
- Throttle/Altitude (Z-axis): Controlled by increasing or decreasing all motors equally
- Forward/Backward (Y-axis): Controlled by pitching the drone (tilting forward/backward)
- Left/Right (X-axis): Controlled by rolling the drone (tilting left/right)
- Rotation around three axes:
- Pitch: Rotation around the X-axis (nose up/down)
- Roll: Rotation around the Y-axis (tilt left/right)
- Yaw: Rotation around the Z-axis (rotate clockwise/counterclockwise)
Control Mechanisms
The flight controller achieves control by varying the speed of individual motors:
Throttle Control
- All motors increase or decrease speed equally
- Directly controls vertical acceleration
- In stable hover, total thrust equals weight
Pitch Control
- Front motors decrease while rear motors increase (or vice versa)
- Creates a torque around the Y-axis
- Results in forward or backward tilt and subsequent movement
Roll Control
- Left motors decrease while right motors increase (or vice versa)
- Creates a torque around the X-axis
- Results in left or right tilt and subsequent movement
Yaw Control
- CW motors increase while CCW motors decrease (or vice versa)
- Creates a net torque around the Z-axis
- Results in clockwise or counterclockwise rotation
I've found that understanding these control mechanisms is crucial for diagnosing flight issues. For example, if your drone drifts right despite level trim, it could indicate a calibration issue, motor power imbalance, or even a subtle frame bend affecting the left-right thrust balance.
Flight Dynamics and Stability
Static vs. Dynamic Stability
Multirotors exhibit different stability characteristics:
- Static Stability: The tendency to return to equilibrium when disturbed
- Multirotors are inherently statically unstable without electronic assistance
- Center of gravity below the propeller plane improves static stability
- Dynamic Stability: The tendency for oscillations to dampen over time
- Affected by PID tuning and control loop performance
- Influenced by frame rigidity and mass distribution
Unlike fixed-wing aircraft that can be designed with inherent stability, multirotors rely entirely on active electronic stabilization.
Moments of Inertia
The mass distribution of a drone significantly affects its flight characteristics:
- Moment of Inertia: Resistance to angular acceleration around an axis
- Centralized Mass: Lower moment of inertia, more responsive to control inputs
- Distributed Mass: Higher moment of inertia, more stable but less responsive
This is why racing drones typically have compact, centralized designs for maximum agility, while photography platforms often have more distributed weight for smoother movement.
The Pendulum Effect
The position of the center of gravity relative to the propeller plane creates a pendulum effect:
- CG below prop plane: Creates a self-righting pendulum effect
- CG at prop plane: Neutral stability, most responsive
- CG above prop plane: Inverted pendulum, highly unstable
Most drones are designed with the battery mounted below the frame to lower the CG and improve stability. I've experimented with different battery positions and found that raising the battery closer to the prop plane increases agility but requires more pilot skill and better tuning.
Control Systems and Flight Controllers
The Control Loop
Modern flight controllers use a feedback control system:

- Setpoint: The desired state (from receiver inputs)
- Feedback: The actual state (from sensors)
- Error: The difference between setpoint and feedback
- Controller: Calculates outputs to minimize error
- Plant: The drone itself, responding to outputs
- Sensors: Measure the drone's state to provide feedback
This closed-loop system runs hundreds of times per second to maintain stability.
PID Control
The most common control algorithm used in flight controllers is PID (Proportional-Integral-Derivative):
- Proportional (P): Responds proportionally to the current error
- Higher P: More aggressive response, potential for oscillation
- Lower P: Smoother response, potential for sluggishness
- Integral (I): Responds to accumulated error over time
- Higher I: Better at correcting persistent errors, potential for overshoot
- Lower I: Less overshoot, may not fully correct errors
- Derivative (D): Responds to the rate of change of error
- Higher D: Better dampening of oscillations, sensitive to noise
- Lower D: Less sensitive to noise, may allow oscillations

Proper PID tuning is essential for optimal flight performance. I typically start with P terms to get a responsive but not oscillating behavior, then add I to eliminate drift, and finally add D to dampen any remaining oscillations.
Gyroscopes and Accelerometers
The primary sensors in a flight controller are:
- Gyroscope: Measures angular velocity around each axis
- Primary sensor for stabilization
- High update rate (8kHz+)
- Subject to drift over time
- Accelerometer: Measures linear acceleration including gravity
- Used for horizon reference
- Lower update rate than gyro
- Affected by drone acceleration
The flight controller fuses data from these sensors to determine the drone's attitude and movement.
Complementary and Kalman Filters
To overcome the limitations of individual sensors, flight controllers use sensor fusion algorithms:
- Complementary Filter: Simple approach that combines gyro and accelerometer data
- Gyro data for short-term accuracy
- Accelerometer data for long-term reference
- Computationally efficient
- Kalman Filter: More sophisticated approach
- Statistical prediction of true state
- Accounts for sensor noise and uncertainty
- More computationally intensive
These filters provide a more accurate estimate of the drone's attitude than any single sensor could provide.
Advanced Flight Concepts
Thrust-to-Weight Ratio
The thrust-to-weight ratio (TWR) is a critical metric for drone performance:
- TWR < 1: Cannot lift off
- TWR ≈ 1-1.5: Slow, stable flight (photography platforms)
- TWR ≈ 2-3: Balanced performance (freestyle drones)
- TWR ≈ 4-7: High-performance (racing drones)
- TWR > 7: Extremely aggressive (specialized racing/freestyle)
Higher TWR provides:
- Better acceleration
- Steeper climb angles
- More aggressive maneuvers
- Shorter stopping distances
- Greater wind resistance
However, it also results in:
- Reduced flight time
- More challenging control
- Higher power requirements
Propeller Disc Loading
Disc loading is the ratio of weight to the total area swept by propellers:
- Low disc loading: More efficient, better hover performance
- High disc loading: Less efficient, better for forward flight
This concept explains why larger props are more efficient for hovering and why increasing prop size often yields better flight time than increasing battery capacity.
Motor Torque and Propeller Inertia
The interaction between motor torque and propeller inertia affects throttle response:
- Motor Torque: Ability to change RPM quickly
- Higher KV motors generally have lower torque
- Larger stator volumes provide more torque
- Propeller Inertia: Resistance to RPM changes
- Larger props have higher inertia
- Heavier props have higher inertia
The ratio between motor torque and propeller inertia determines throttle responsiveness. This is why racing drones often use smaller, lighter props with high-torque motors for maximum responsiveness.
Throttle Linearity and Resolution
The relationship between throttle input and thrust output is non-linear:
- Thrust increases with the square of motor RPM
- This creates a non-linear throttle response
- Digital protocols like DShot help improve resolution
- Modern flight controllers implement throttle curves to linearize response
Understanding this non-linearity is crucial for precise throttle control, especially in proximity flying and racing scenarios.
Ground Effect
When flying close to a surface, drones experience the ground effect:
- Increased efficiency when hovering within ~1 prop diameter of a surface
- Created by cushioning of air between propellers and surface
- Can cause unexpected lift when taking off or landing
- More pronounced with larger propellers
I've noticed this effect is particularly strong with cinematic drones using large props, sometimes causing them to "bounce" during landing attempts if throttle isn't reduced appropriately.
Flight Modes and Control Algorithms
Rate Mode vs. Self-Leveling
Modern flight controllers offer different control modes:
- Rate Mode (Acro/Manual):
- Controller only stabilizes rotation rates
- Drone will maintain its attitude when sticks are centered
- Allows for complete freedom of movement including inverted flight
- Preferred by freestyle and racing pilots
- Self-Leveling Modes (Angle/Horizon):
- Controller actively works to maintain level orientation
- Drone returns to level when sticks are centered
- Limited maximum tilt angle
- Easier for beginners and aerial photography
The key difference is that rate mode controls angular velocity, while self-leveling modes control absolute angle.
Flight Envelope Protection
Some flight controllers implement limits to prevent dangerous situations:
- Angle limits: Prevent excessive tilt that could cause loss of altitude
- Power limits: Prevent battery damage from excessive current draw
- Velocity limits: Restrict maximum speed for safety
- Geofencing: Prevent flying in restricted areas or beyond a set distance
These protections are more common in commercial and photography drones than in racing/freestyle models.
Advanced Control Algorithms
Beyond basic PID, advanced flight controllers implement:
- Feedforward: Anticipates control needs based on stick input
- Dynamic notch filters: Automatically identify and filter out resonant frequencies
- Anti-gravity: Compensates for thrust changes during rapid throttle adjustments
- Airmode: Maintains authority during zero-throttle maneuvers
These algorithms significantly improve flight performance, particularly in extreme maneuvers and racing scenarios.
Environmental Factors Affecting Flight
Air Density and Altitude
Air density directly affects propeller performance:
- Higher density (lower altitude, colder air): More thrust, better efficiency
- Lower density (higher altitude, warmer air): Less thrust, reduced efficiency
At 5000 feet above sea level, a drone might lose 10-15% of its thrust compared to sea level performance. I've experienced this firsthand when flying in mountain locations, where drones feel noticeably less powerful and responsive.
Wind and Turbulence
Wind creates complex challenges for drone flight:
- Steady wind: Requires constant correction to maintain position
- Gusting wind: Unpredictable disturbances requiring rapid response
- Turbulence: Chaotic air movement, especially around obstacles
- Wind gradient: Wind speed increases with height above ground
Modern flight controllers can compensate for wind to some extent, but understanding wind patterns and limitations is essential for safe flying.
Temperature Effects
Temperature affects multiple aspects of drone performance:
- Motors: Higher temperatures reduce efficiency and maximum power
- Batteries: Cold reduces capacity and voltage; heat increases internal resistance
- Electronics: Extreme temperatures can affect sensor calibration
- Air density: Higher temperatures reduce air density and thrust
I've found that performance in very cold conditions (below freezing) can drop by 20-30% compared to optimal temperatures, primarily due to battery limitations.
FAQ: Common Questions About Drone Flight Theory
How do drones maintain stability without a tail rotor like helicopters?
Unlike helicopters that use a tail rotor to counteract main rotor torque, multirotors use counter-rotating propellers. In a quadcopter, two motors spin clockwise and two spin counterclockwise, balancing the torque forces. This design eliminates the need for a tail rotor and simplifies the mechanical system significantly. The flight controller constantly adjusts individual motor speeds to maintain the desired orientation.
What's the difference between brushed and brushless motors in terms of flight dynamics?
Brushless motors provide higher power-to-weight ratios, better efficiency, and faster response times compared to brushed motors. From a flight dynamics perspective, brushless motors offer:
- More precise control due to faster acceleration/deceleration
- Higher maximum thrust for better performance
- More consistent performance throughout the battery discharge cycle
- Lower moment of inertia in the rotating assembly
These advantages translate to more responsive flight characteristics and better overall performance, which is why virtually all performance drones use brushless motors.
How does battery voltage affect flight characteristics?
Battery voltage has several effects on flight:
- Higher voltage allows motors to spin faster, increasing maximum thrust
- Voltage sag under load affects available power during aggressive maneuvers
- Voltage decreases as the battery discharges, gradually reducing performance
- Different voltage systems (3S, 4S, 6S) require appropriate motor KV selection
Modern flight controllers implement voltage compensation to maintain consistent flight characteristics throughout the battery discharge cycle, but the maximum performance will still decrease as voltage drops.
Why do racing drones use such high tilt angles?
Racing drones often fly at extreme forward tilt angles (30-60°) for several reasons:
- To direct more thrust forward for acceleration and speed
- To reduce frontal area and minimize drag
- To maintain line-of-sight with the racing gates/obstacles below
The maximum efficient tilt angle depends on the thrust-to-weight ratio. A drone needs a TWR of at least 1.15 to hover at a 30° angle, 1.41 to hover at 45°, and 2.0 to hover at 60°. Racing drones typically have TWRs of 4-7+ to allow for these extreme angles while maintaining altitude and acceleration capability.
How do altitude and air density affect drone performance?
As altitude increases, air density decreases, which reduces propeller efficiency. This affects drones in several ways:
- Reduced maximum thrust (typically 3-4% per 1000ft of elevation)
- Decreased control authority and responsiveness
- Higher motor temperatures due to less cooling
- Potentially shorter flight times due to motors working harder
When flying at high altitudes, it's advisable to use larger propellers, higher capacity batteries, and more conservative flight patterns to compensate for these effects.
What causes propwash oscillations and how can they be minimized?
Propwash oscillations occur when a drone flies through the turbulent air created by its own propellers, typically during quick direction changes or descents. This disturbed airflow reduces propeller efficiency and creates unpredictable forces that the flight controller must compensate for.
To minimize propwash:
- Tune PID values appropriately, particularly D terms
- Use dynamic filtering to adapt to changing conditions
- Implement advanced flight controller features like propwash mitigation
- Adopt flying techniques that minimize rapid transitions through disturbed air
- Consider frame designs that separate props further or use ducts
How does weight distribution affect flight characteristics?
Weight distribution determines the drone's moments of inertia around each axis, which directly affects how quickly it can change orientation:
- Centralized mass (lower moment of inertia) allows faster rotation rates
- Distributed mass (higher moment of inertia) provides more stable, smooth movement
- Asymmetrical weight distribution can cause inconsistent handling between axes
Racing drones typically centralize mass for maximum agility, while photography platforms distribute weight more evenly for smoother movement and better stability.
What's the relationship between motor KV, voltage, and propeller size?
These three factors must be balanced for optimal performance:
- Motor KV determines RPM per volt (e.g., a 2400KV motor on 4S will spin at approximately 35,520 RPM unloaded)
- Higher KV motors work best with smaller propellers and lower voltages
- Lower KV motors work best with larger propellers and higher voltages
The ideal combination provides the desired thrust while keeping motors within their efficient operating range. As a general rule:
- 5" props: 1800-2600KV on 4S, 1300-1700KV on 6S
- 6" props: 1600-1900KV on 4S, 1100-1400KV on 6S
- 7" props: 1300-1600KV on 4S, 900-1200KV on 6S
How do gyro filters affect flight performance?
Gyro filters remove noise from sensor data but introduce latency:
- More filtering provides smoother operation but slower response
- Less filtering provides faster response but may allow noise to affect control
- Dynamic filters automatically adjust to current conditions
The optimal filtering balance depends on the application:
- Racing: Minimal filtering for maximum responsiveness
- Freestyle: Moderate filtering for clean response without oscillations
- Photography: Higher filtering for smooth, predictable movement
What causes battery voltage sag and how does it affect flight?
Voltage sag occurs when a battery cannot maintain its voltage under load due to internal resistance:
- More pronounced with older or lower-quality batteries
- Worsens as the battery discharges
- Increases with higher current draw (aggressive flying)
This voltage drop reduces available power and can cause:
- Reduced maximum thrust during high-throttle maneuvers
- Inconsistent flight characteristics
- Premature low-voltage warnings
- Potential loss of control if severe
Using batteries with appropriate C-ratings for your flying style and implementing throttle limiting as batteries discharge can help manage these effects.
Conclusion
Understanding the theoretical principles behind multirotor flight provides a foundation for becoming a more skilled pilot, builder, and troubleshooter. While modern flight controllers handle the complex calculations needed for stable flight, knowing how and why drones behave as they do allows you to:
- Make informed component choices for your specific needs
- Tune your drone for optimal performance
- Diagnose and solve flight issues
- Fly with greater precision and confidence
- Push the boundaries of what's possible with multirotor aircraft
The field of drone technology continues to evolve rapidly, with advances in motors, batteries, control algorithms, and materials constantly expanding the performance envelope. By understanding the fundamental principles covered in this guide, you'll be well-equipped to evaluate and leverage these innovations in your own flying.
Whether you're building your first drone or your hundredth, I hope this guide helps deepen your understanding of the fascinating physics and engineering that make multirotor flight possible. Happy flying!
References and Further Reading
- Drone Motors: Selection, Performance, and Optimization
- Drone Propellers: Selection, Performance, and Optimization
- Drone Frames: Sizes, Materials, and Selection
- Drone Flight Controllers: Principles and Operation
- Drone Vibration Damping
Comments ()